Advanced spectroscopy techniques developed by MIT Chemistry Professor Keith A. Nelson are yielding insights into heat transfer in crystalline materials that overturn long-held assumptions.
Nelson’s work could lead to the engineering of materials with tailored heat-transfer properties for use in semiconductors, thermoelectrics, and other applications where managing heat is important.
When heat travels through a solid, it is carried by acoustic phonons, which are the individual quanta of energy in an acoustic wave, just as photons are for a light wave. Phonon travel is limited by the speed of sound, which is 10,000 times slower than the speed of light. Acoustic waves for heat transport — in the range of 0.1 to 10 terahertz — are millions of times higher than the range of human hearing.
But Nelson and colleagues have developed time-resolved optical spectroscopy methods for measuring the acoustic waves associated with heat transfer.
Alternating heated and unheated regions
The technique crosses two laser beams to form an interference "grating" pattern that creates alternating heated and unheated sections in the target material. Heat from absorption of the crossed laser beams expands the material in the grating pattern, which allows the pattern to diffract probe laser light, so measurement of the diffracted signal detects the spatially varying temperature changes.
"The material is less dense where it was heated because it expanded, and there was some contraction because of that in the unheated regions, so the density now is modulated with the exact same pattern,” Nelson says. Thermal transport from the heated to the unheated regions washes out the pattern, so a gradual time-dependent decay of diffracted signal is observed. For a pattern with a particular spatial period, the measurement reveals how long heat transfer takes.
A collaborative effort through the MIT-based Solid State Solar Thermal Energy Conversion Center (S3TEC, a DOE-funded Energy Frontier Research Center) that included staff scientist Alexei Maznev and fourth-year chemistry graduate student Jeffrey K. Eliason demonstrated experimentally that in silicon at room temperature, the long-held diffusive model of heat transfer was invalid for small distances from 1 to 5 microns, where ballistic movement seems to be the driving force. (See related article.)
Random walk vs. ballistic transport
The old model was based on the assumption that phonons diffuse in a solid the way that molecules of a spray from a perfume bottle disperse in air. “By the time it gets to you several feet away, the molecules from the bottle did not go directly from the bottle to your nose in a straight unwavering path,” Nelson explains. “They got diverted and bounced back and forth all over the place, and they managed to scatter and randomly drift over to you. That’s what leads to the motion that we call diffusion.” The phenomenon is also known as random walking. Similarly, scientists had thought heat transport in a solid was governed by thermal diffusion. That assumption was based on an average phonon mean free path in silicon of about 40 nanometers.
“But it turns out when we made measurements of the thermal transport, and this was in crystalline silicon, at room temperature, later in gallium arsenide, even above room temperature, that’s not what we see,” Nelson says. Instead, the researchers saw what looked like a significant component of straight-line, ballistic motion. “Once we start getting to 20, 15, 10 microns, 5 microns, that stops, and what’s happening is, at those length scales, we no longer have purely diffusional motion. And what that’s telling us is that the things that are carrying the heat, which are these acoustic waves, are not scattering lots and lots of times, on that length scale. They’re moving ballistically.”
“That may seem like a short distance, but that’s macroscopic,” Nelson says. “I can see it in a microscope. It’s not nanometers, and in particular, when we build devices, we worry about how transport works. In materials like thermoelectrics, we care about motion on length scales like that. So we can’t model it as diffusion. The thermal conductivity that we would get is really way off if we try to do that.”
Directly measuring acoustic phonon mean free paths
Nelson hopes to directly measure the acoustic phonon mean free paths by optically generating acoustic waves and monitoring them. “We’re working hard to be able to measure acoustic waves at the highest frequencies, these terahertz-frequency acoustic waves, in different materials for this thermal transport work, and it’s hard,” Nelson says. “We don’t have very good ways of using our excitation light and turning it into terahertz-frequency acoustic waves. We’re pretty good at megahertz and gigahertz frequencies, not these highest frequencies and shortest wavelengths.” He hopes to take terahertz-frequency light waves, convert them into terahertz-frequency acoustic waves, then reconvert the terahertz-frequency acoustic wave to a terahertz-frequency light wave, and detect that. “We’re not there yet; we’re close,” he says.
Those next steps will require extremely flat interfaces and high frequencies, because the wavelengths involved will only be as short as the thickness of the interface where it happens, Nelson says. “If I have an atomically flat interface, which I can get in some materials, then I can make the very shortest acoustic wavelengths — only a couple of unit cells in length. That’s what we’re trying to do and hoping that we’ll be able to do. And it’s really only possible because we now have strong enough terahertz electromagnetic waves that we have real hope of first converting them into acoustic waves — the efficiency of that isn’t high — then reconverting those back into terahertz electromagnetic waves, again for which the efficiency isn’t high, and still detecting that at the end of the day. If I want that to work, I’d better start with a fairly strong terahertz wave, so that that weak terahertz wave I have at the end is still detectable. I think we have what we need in order to make that happen.”
Reaching out to high schools
Nelson also leads an outreach program to area high school students, the Lambda Project, which brings groups of four students at a time to the spectroscopy lab for three days. Students learn how a thin-film sample is made by deposition in the MIT Chemistry undergraduate labs. They also learn how to operate the spectroscopy equipment, take measurements, and analyze the data. Swampscott High School teacher Jill Sewell currently is lab manager for the program. (See related article.)
“There is a sound wave, and there’s thermal expansion, and students measure it,” Nelson says. “At a more basic level, they’re learning about nanometers and microns, for the nanometer-thickness films and micron interference spacing and so forth, and they’re learning about picoseconds and nanoseconds. For a high school student, what these length and time scales are, and what sorts of things happen or are built on those time scales or length scales, are new. There is a lot of basic learning through exposure to things they are not normally seeing.”
Spectroscopy techniques demonstrate ballistic motion at micron length scales, open door to new possibilities for semiconductors, thermoelectrics.
Publication Date:
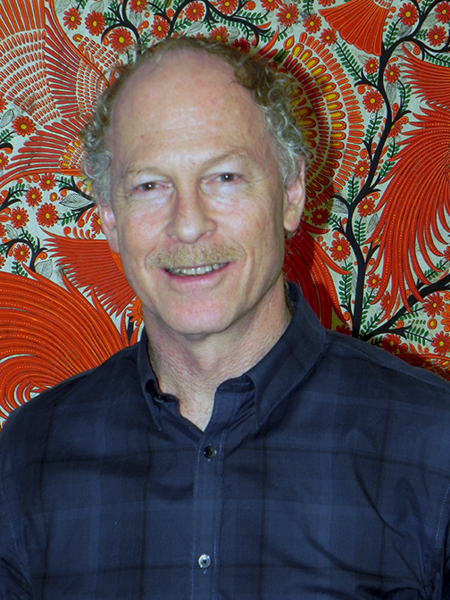
Caption:
MIT Chemistry Professor Keith A. Nelson
Credits:
Denis Paiste, Materials Processing Center